The cosmic story that unfolded following the Big Bang is ubiquitous no matter where you are. The formation of atomic nuclei, atoms, stars, galaxies, planets, complex molecules, and eventually life is a part of the shared history of everyone and everything in the Universe. Even though all of these things likely arise at somewhat different times at different locations in the Universe, largely dependent on the initial conditions such as temperature and density, once enough time goes by, they’re found literally everywhere. At least once, here on Earth, life began at some point in the Universe. At the absolute latest, it appeared only a few hundred million years after our planet was first formed.
That puts life as we know it arising, at the absolute latest, nearly 10 billion years after the Big Bang. When the Big Bang first occurred, life was impossible. In fact, the Universe couldn’t have formed life from the very first moments; both the conditions and the ingredients were all wrong. But that doesn’t mean it took all those billions and billions of years of cosmic evolution to make life possible. Based on when the raw ingredients that we believe are necessary for the most primitive forms of life to arise from non-life, it’s reasonable to think that “first life” might have come around back when the Universe was just a few percent of its current age. Here’s the best scientifically-motivated story for how life might have first arisen in our Universe.
The existence of complex, carbon-based molecules in star forming regions is interesting, but isn’t anthropically demanded. Here, glycolaldehydes, an example of simple sugars, are illustrated in a location corresponding to where they were detected in an interstellar gas cloud: offset from the region presently forming new stars the fastest. Interstellar molecules are common, with many of them being complex and long-chained.
At the earliest moments of the hot Big Bang, the raw ingredients for life could in no way stably exist. Particles, antiparticles, and radiation all zipped around at relativistic speeds, blasting apart any bound structures that might have formed by chance. As the Universe aged, though, it also expanded and cooled, reducing the kinetic energy of everything in it. Over time, antimatter annihilated away, stable atomic nuclei formed, and electrons finally bound to them, forming the first neutral atoms in the Universe.
Yet these earliest atoms were only hydrogen and helium: insufficient for life. Heavier elements, such as carbon, nitrogen, oxygen and more, are required to build the molecules that all life processes rely on. For that, we need to form stars in great abundance, have them go through their life-and-death cycle, and return the products of their nuclear fusion to the interstellar medium.
It takes 50-to-100 million years to form the first stars, sure, which form in relatively large clusters. But in the densest regions of space, these star clusters will gravitationally pull in other matter, including material for additional stars and other star clusters, paving the way for the first galaxies. By time only ~200-to-250 million years have passed, not only will multiple generations of stars have lived-and-died, but the earliest star clusters will have grown into galaxies.
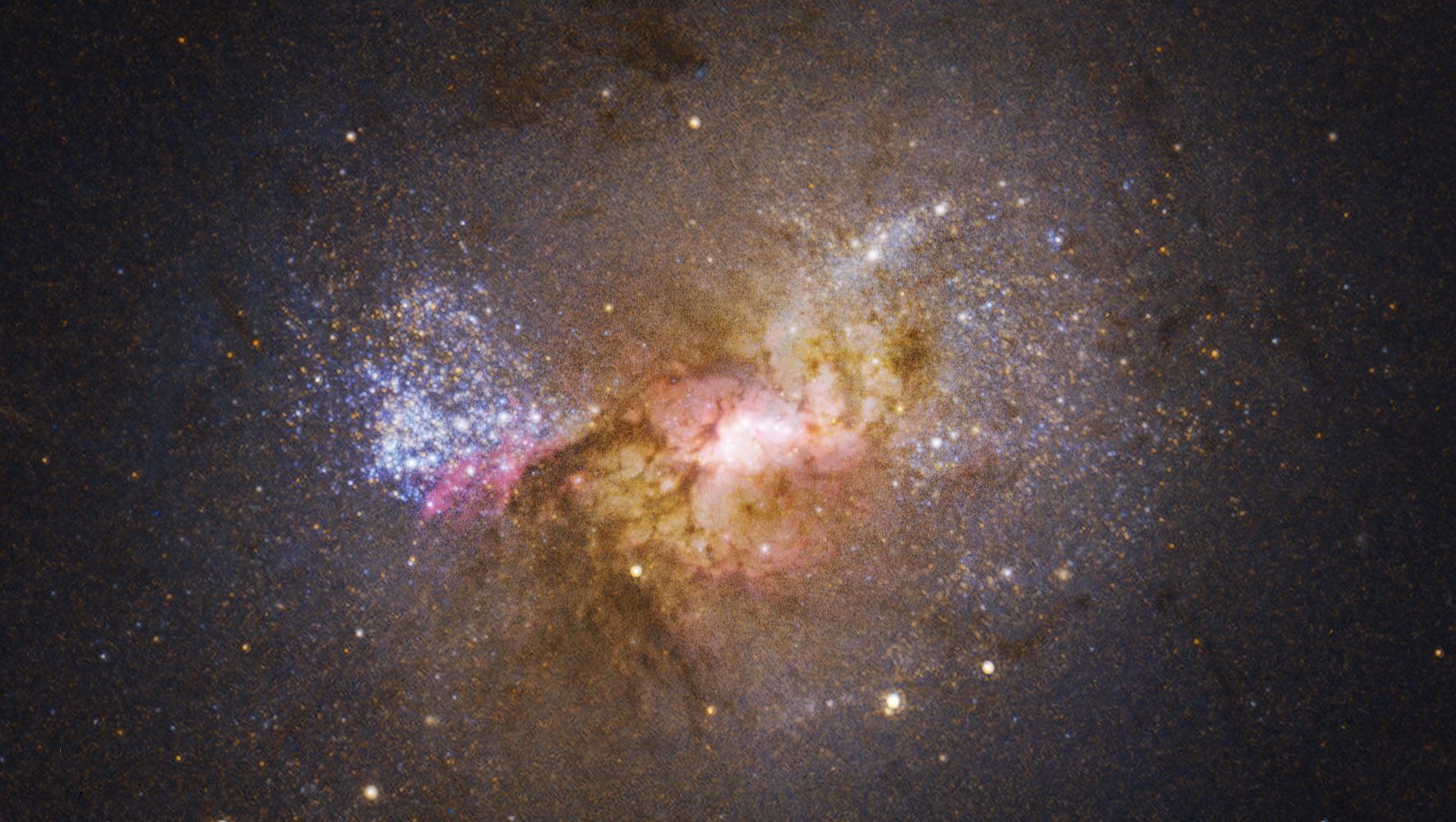
When the very first stars form in the Universe, they form out of hydrogen and helium alone. But when that first generation dies, it can give rise to a second generation that’s far more complex, intricate, and diverse. The resulting starburst from the merger of multiple early, merging star clusters may resemble Henize 2-10, a nearby star-forming galaxy located 30 million light years away. Once a sufficient quantity of heavy elements builds up, the formation of rocky planets, and the potential for life arising on them, can no longer be ignored.
This is important, because we don’t just need to create the heavy elements like carbon, nitrogen, and oxygen; we need to create enough of them — and all of the life-essential elements — to produce a wide diversity of organic molecules.
We need those molecules to stably exist in a location where they can experience an energy gradient, such as on a rocky moon or planet in the vicinity of a star, or with enough undersea hydrothermal activity to support certain chemical reactions. And we need for those locations to be stable enough that whatever counts as a life process can self-sustain.
In astronomy, all of these conditions get lumped together under the umbrella of a single term: metals. A “metal,” to an astronomer, is any element heavier than hydrogen or helium, from lithium (element #3) all the way up as high as the periodic table can theoretically go. Whenever we look at a star, we can measure the strength of the different absorption lines coming from it, which tell us — in combination with the star’s temperature and ionization — what the abundances of the different elements are that went into creating it. Add them all up, and that gives you the star’s metallicity, or the fraction of the elements within it that are heavier than either plain hydrogen or helium.

What do planets outside our solar system, or exoplanets, look like? A variety of possibilities are shown in this illustration. Scientists discovered the first exoplanets in the 1990s. As of 2023, the tally stands at just over 5,000 confirmed exoplanets. None are known to be inhabited, but a few raise tantalizing possibilities: largely among the Earth-sized planets, not the super-Earth-sized ones.
Our Sun’s metallicity is somewhere between 1-and-2%, but that appears to be too excessive for a requirement for life. Stars possessing just a fraction of the heavy elements (metals) found in the Sun and the rest of the Solar System, might still have enough of the necessary ingredients, across-the-board, to make life possible.
Remarkably, we’ve detected more than 5000 exoplanets over the past ~20 years, and there are tremendous lessons to learn from the stellar systems that we do and don’t find the “rocky” ones in. In particular:
- Only 10 exoplanets orbit stars with 10% or fewer of the heavy elements found in the Sun.
- Only 32 exoplanets orbit stars with between 10% and 16% of the Sun’s heavy elements.
- And only 50 exoplanets orbit stars with between 16% and 25% of the Sun’s heavy elements.
That means, all told, that only 92 out of the 5069 exoplanets found as of early 2023 — just 1.8% — exist around stars with a quarter or fewer of the heavy elements found in the Sun. In other words, if you want to make rocky planets, the ones that we think support life, you need to enrich the interstellar medium sufficiently, and that takes time.
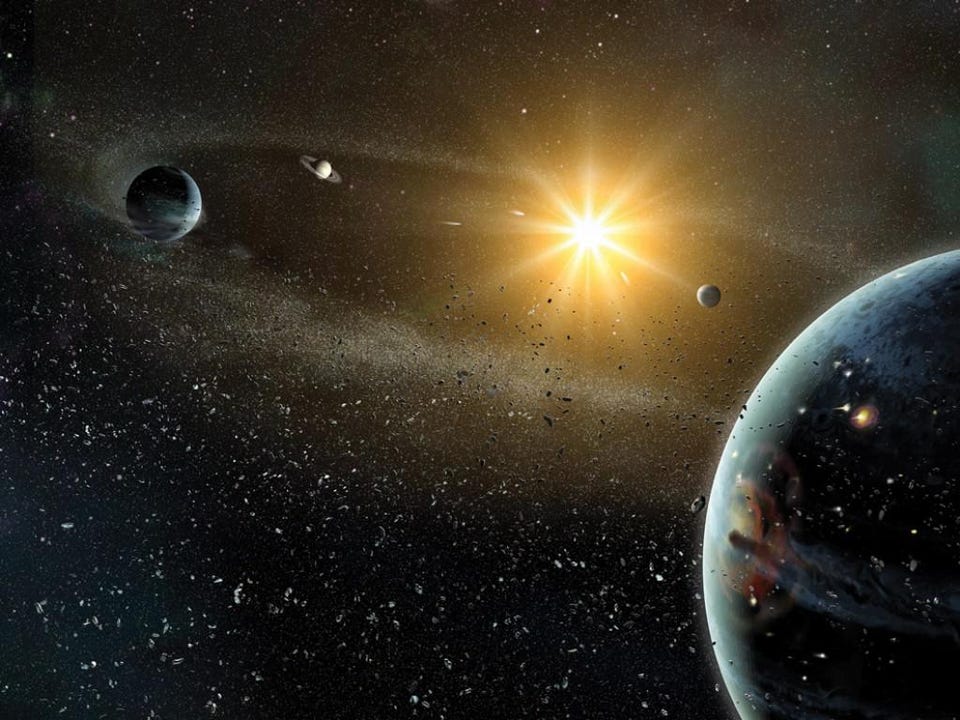
Long after the planet-forming gas from a protoplanetary system is blown away, large quantities of dusty debris can persist, bombarding the young planetary system for several hundreds of millions of years. This happened in our early Solar System for about ~600 million years, and is still potentially happening around stars such as Vega, Fomalhaut, and Epsilon Eridani. The evidence for first life on Earth can be no more than perhaps 100 million years after the end of this period, and may have even begun during the heavy bombardment phase of our planet’s history, with incredible implications for life elsewhere in the Universe.
However, remember what’s happening in the Universe as far as stars are concerned: they’re forming from very early times onwards, and the star-formation rate, even though it starts small, continually increases over the first ~3 billion years of cosmic history. As more stars form from the ashes of older stars that have lived-and-died, the heavy element content, as well as the probability of forming stellar systems that will possess rocky planets, increases as time goes on. While most stars won’t form with rocky planets around them until several billion years have passed since the Big Bang, the first ones to get there might take only around one billion years: the first truly hospitable locations for life to arise in the cosmos.
The big question, then, becomes “how?” How did life arise? What are the conditions that support its creation from non-life, what were the specific mechanism(s) that allowed it to happen, and in the places where life managed to sustain itself, i.e., to survive and reproduce and thrive for generation after generation, what were the conditions that arose that enabled a long-term unbroken chain of biological activity? Even though we haven’t figured out the answer to those questions as far as Earth’s own history is concerned, we’ve made tremendous advances in recent years, particularly on the “mechanism” side for life arising from non-life.

Early on, shortly after the Earth first formed, life likely arose in the waters of our planet. The evidence we have that all life that’s extant today can be traced back to a universal common ancestor is very strong, but the early stages of our planet, for perhaps the first 1-to-1.5 billion years, remains largely obscure.
The best proxy for understanding where the ingredients for life came from is to simply look at the composition of the asteroids and comets we find in space, as well as the remnants of meteorites that have survived their journey down to Earth today. When we look inside these primitive objects, many of which we can use atomic technique to date back to ~4.56 billion years ago, we find:
While there are some who claim that these ingredients, if you smash them all together in a primordial soup (i.e., an aqueous environment with an energy gradient), may have given rise to self-replicating life spontaneously, that’s by far a minority opinion. Instead, a vastly preferred pathway by almost all working biologists is the idea that the ability to metabolize something of nutritional value is what came first.

Deep under the sea, around hydrothermal vents, where no sunlight reaches, life still thrives on Earth. How to create life from non-life is one of the great open questions in science today, but hydrothermal vents are one of the leading locations where the first metabolic processes, the precursor to living organisms, may have first arisen. If life can exist down there on Earth, perhaps undersea on Europa or Enceladus, there’s life down there, too.
Let’s imagine what this might have looked like. On any world with enough liquid water, there will be plenty of naturally occurring aqueous environments:
- the salty oceans and tidepools,
- freshwater sources like lakes and rivers,
- or even subsurface oceans that persist underneath rocky or icy crusts.
There will also be sources of external energy in the form of sunlight and geothermal heat, including in deep sea vents and along hydrothermal fields. There will be minerals and ions dissolved in that water, as well as all sorts of molecules, including a wide variety of amino acids that can bind together. And, perhaps most importantly from a thermodynamic perspective, you have chemical non-equilibrium states at a wide variety of interfaces: solid earth/liquid water, liquid water/volcanic magma, and liquid water/atmospheric gas.
As amino acids smack into each other, they spontaneously form and break bonds, with chains of amino acids forming peptides. As ions come along and bind to these primitive peptides, they enable the creation of enzymes. These molecules are fragile and easy to destroy or denature, but they’re also very large in number and the possibilities — set by the so-large-it’s-barely-fathomable mathematics of combinatorics — boggle the mind. Some of the proteins that form, merely by chance, will gain the ability to perform specific functions. These functions might have included:
- the gathering or even the hoarding of resources, including specific peptides,
- the ability to split/recombine other molecules in a way that liberates usable energy in the process,
- and the ability to “bite” other useful molecules, while remaining intact themselves.
Whatever the case, the spontaneous creation of these metabolic peptides are all but inevitable. What comes next, fascinatingly, is a brand new but startling area of research.

If life began with a random peptide that could metabolize nutrients/energy from its environment, replication could then ensue from peptide-nucleic acid coevolution. Here, DNA-peptide coevolution is illustrated, but it could work with RNA or even PNA as the nucleic acid instead. Asserting that a “divine spark” is needed for life to arise is a classic “God-of-the-gaps” argument.
It has recently been shown that if you have nucleobases in an aqueous environment — things like RNA, DNA, or even PNA (peptide nucleic acids) — that these nucleotides will line up along the various amino acids in a peptide chain. If they can pair up with their conjugate base, or “peel off” and draw additional amino acids onto them, they can effectively reproduce, to a high degree of accuracy, the original peptide chain.
This scenario, known as RNA-peptide coevolution, is how most working scientists investigating the origin of life now believe that self-replication, built on the backbone of metabolic processes, first came about.
Although not every biologist agrees that:
- a free-floating molecule,
- that can metabolize resources,
- and replicate itself,
rises to the threshold of being “life” rather than “non-life,” this likely represents the first concrete steps that led from simple chemical processes to biological ones. These primitive “metabolizing replicators” likely came into existence alongside one another, possessing a great diversity among them, with many — if not most — of them certainly going extinct along the way. This predates a universal common ancestor on Earth, and even our notion of what a cell is, by many hundreds of millions (and maybe over a billion) years. Nonetheless, this is where current scientific thought takes us as to how life first originated on Earth.
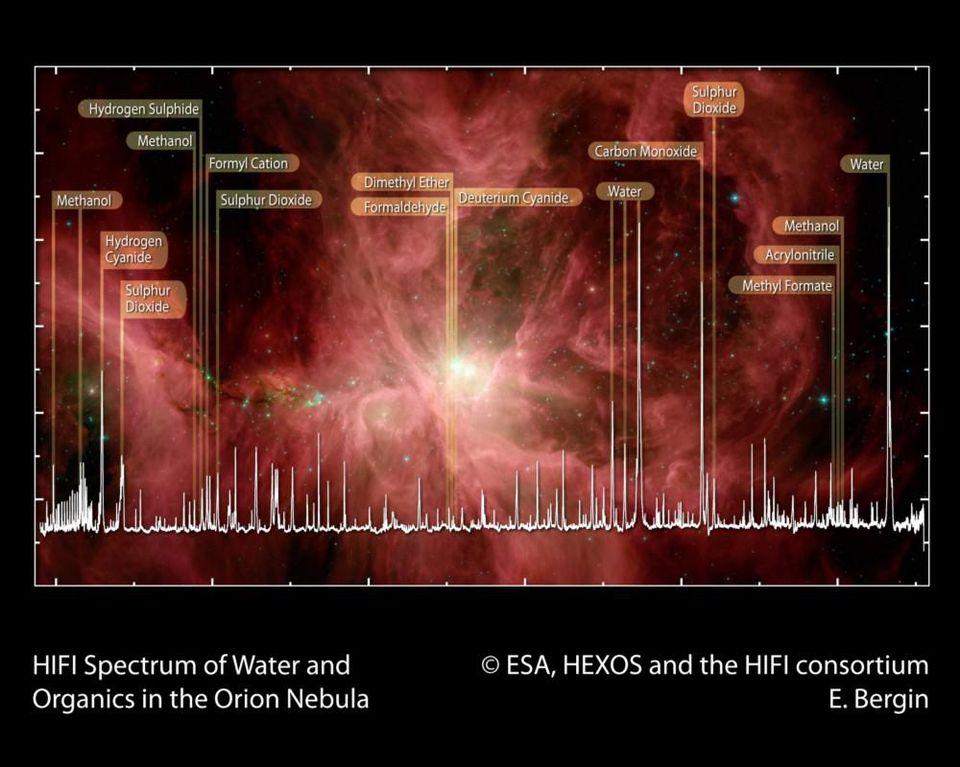
The raw ingredients that we believe are necessary for life, including a wide variety of carbon-based molecules, are found not only on Earth and other rocky bodies in our Solar System, but in interstellar space, such as in the Orion Nebula: the nearest large star-forming region to Earth.
Because we have every reason to believe that the laws and ingredients we have on Earth are found all over the Universe, it makes sense to look for those same “fingerprints” wherever we’re capable of looking. In space, whether around the centers of galaxies or around massive, newly forming stars, or even in the environments where metal-rich gas is going to form future stars, we find a whole host of complex, organic molecules. These range from sugars to amino acids to ethyl formate (the molecule that gives raspberries their scent) to intricate aromatic hydrocarbons; i.e., molecules that are thought to be precursors to life.
Travel the Universe with astrophysicist Ethan Siegel. Subscribers will get the newsletter every Saturday. All aboard!
So far, we’ve only found these molecular “bio-hints” nearby, of course, but that’s because we don’t know how to look for individual molecular signatures in environments that lie well beyond our own galaxy. However, as we look to greater and greater distances, we do indeed find that there are galaxies and portions of even very early galaxies that have the right populations of stars and the right metallicities to them to be excellent candidates for life to arise within them. In the most extreme cases, we find locations from within the first 1-2 billion years after the Big Bang that might potentially be home to life already.

This infrared portrait of the Small Magellanic Cloud, located just 199,000 light-years away, highlights a variety of features, including new stars, cool gas, and quite spectacularly (in green) the presence of polycyclic aromatic hydrocarbons: the most complex organic molecules ever found in the natural environment of interstellar space. The way that atoms link up to form molecules, including organic molecules and biological processes, is only possible because of the Pauli exclusion rule that governs electrons, and happens everywhere across the Universe were enough heavy elements are present.
It has to be said, however, that we still don’t know how life in the Universe (or even on Earth) got its start, including whether life as we know it is common, rare, or a once-in-a-Universe proposition. But we can be certain that life came about in our cosmos at least once, and that it was built out of the heavy elements made from previous generations of stars. If we look at how stars theoretically form in young star clusters and early galaxies, we could reach that abundance threshold after several hundred million years; all that remains is putting those atoms together in a favorable-to-life arrangement.
If the Universe forms the molecules necessary for life and then puts them in an environment conducive to life arising from non-life, like on a water-rich rocky planet, suddenly the emergence of biology could have come when the Universe was just a few percent of its current age. The earliest life in the Universe, we must conclude, could have been possible during even the first one or two billion years after the hot Big Bang began. Once enough stars live-and-die, the material from their corpses gets incorporated into new stars, new molecules, and even new planets. Get enough of this enriched material together under the right conditions, and that’s perhaps all it takes to result in life’s all-but-guaranteed arrival.

Dr. Thomas Hughes is a UK-based scientist and science communicator who makes complex topics accessible to readers. His articles explore breakthroughs in various scientific disciplines, from space exploration to cutting-edge research.